Bioengineered constructs combined with exercise enhance stem cell-mediated treatment of volumetric muscle loss
- PMID: 28631758
- PMCID: PMC5481841
- DOI: 10.1038/ncomms15613
Bioengineered constructs combined with exercise enhance stem cell-mediated treatment of volumetric muscle loss
Abstract
Volumetric muscle loss (VML) is associated with loss of skeletal muscle function, and current treatments show limited efficacy. Here we show that bioconstructs suffused with genetically-labelled muscle stem cells (MuSCs) and other muscle resident cells (MRCs) are effective to treat VML injuries in mice. Imaging of bioconstructs implanted in damaged muscles indicates MuSCs survival and growth, and ex vivo analyses show force restoration of treated muscles. Histological analysis highlights myofibre formation, neovascularisation, but insufficient innervation. Both innervation and in vivo force production are enhanced when implantation of bioconstructs is followed by an exercise regimen. Significant improvements are also observed when bioconstructs are used to treat chronic VML injury models. Finally, we demonstrate that bioconstructs made with human MuSCs and MRCs can generate functional muscle tissue in our VML model. These data suggest that stem cell-based therapies aimed to engineer tissue in vivo may be effective to treat acute and chronic VML.
Conflict of interest statement
The authors declare no competing financial interests.
Figures
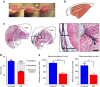
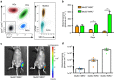
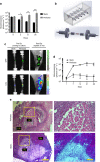
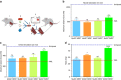
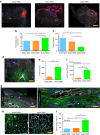
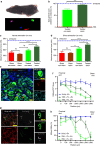
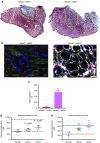
Similar articles
-
Long-Term Evaluation of Functional Outcomes Following Rat Volumetric Muscle Loss Injury and Repair.Tissue Eng Part A. 2020 Feb;26(3-4):140-156. doi: 10.1089/ten.TEA.2019.0126. Epub 2020 Jan 23. Tissue Eng Part A. 2020. PMID: 31578935 Free PMC article.
-
Biomechanics show stem cell necessity for effective treatment of volumetric muscle loss using bioengineered constructs.NPJ Regen Med. 2018 Oct 10;3:18. doi: 10.1038/s41536-018-0057-0. eCollection 2018. NPJ Regen Med. 2018. PMID: 30323949 Free PMC article.
-
Synergistic effect of high-intensity interval training and stem cell transplantation with amniotic membrane scaffold on repair and rehabilitation after volumetric muscle loss injury.Cell Tissue Res. 2021 Feb;383(2):765-779. doi: 10.1007/s00441-020-03304-8. Epub 2020 Oct 31. Cell Tissue Res. 2021. PMID: 33128624
-
The Potential of Combination Therapeutics for More Complete Repair of Volumetric Muscle Loss Injuries: The Role of Exogenous Growth Factors and/or Progenitor Cells in Implantable Skeletal Muscle Tissue Engineering Technologies.Cells Tissues Organs. 2016;202(3-4):202-213. doi: 10.1159/000447323. Epub 2016 Nov 9. Cells Tissues Organs. 2016. PMID: 27825153 Review.
-
Cells, scaffolds, and bioactive factors: Engineering strategies for improving regeneration following volumetric muscle loss.Biomaterials. 2021 Nov;278:121173. doi: 10.1016/j.biomaterials.2021.121173. Epub 2021 Oct 1. Biomaterials. 2021. PMID: 34619561 Free PMC article. Review.
Cited by
-
Biomimetic sponges improve muscle structure and function following volumetric muscle loss.J Biomed Mater Res A. 2021 Nov;109(11):2280-2293. doi: 10.1002/jbm.a.37212. Epub 2021 May 7. J Biomed Mater Res A. 2021. PMID: 33960118 Free PMC article.
-
The mechanosensitive ion channel PIEZO1 promotes satellite cell function in muscle regeneration.Life Sci Alliance. 2022 Nov 29;6(2):e202201783. doi: 10.26508/lsa.202201783. Print 2023 Feb. Life Sci Alliance. 2022. PMID: 36446523 Free PMC article.
-
Amniotic Membrane Scaffolds Support Organized Muscle Regeneration in A Murine Volumetric Muscle Defect Model.Plast Reconstr Surg Glob Open. 2022 Sep 14;10(9):e4499. doi: 10.1097/GOX.0000000000004499. eCollection 2022 Sep. Plast Reconstr Surg Glob Open. 2022. PMID: 36119379 Free PMC article.
-
Contactless magnetically responsive injectable hydrogel for aligned tissue regeneration.Mater Today Bio. 2024 Jun 3;27:101110. doi: 10.1016/j.mtbio.2024.101110. eCollection 2024 Aug. Mater Today Bio. 2024. PMID: 39211510 Free PMC article.
-
Long-Term Evaluation of Functional Outcomes Following Rat Volumetric Muscle Loss Injury and Repair.Tissue Eng Part A. 2020 Feb;26(3-4):140-156. doi: 10.1089/ten.TEA.2019.0126. Epub 2020 Jan 23. Tissue Eng Part A. 2020. PMID: 31578935 Free PMC article.
References
-
- Grogan B. F. & Hsu J. R. Skeletal Trauma Research Consortium. Volumetric muscle loss. J. Am. Acad. Orthop. Surg. 19, S35–S37 (2011). - PubMed
-
- Garg K. et al.. Volumetric muscle loss: persistent functional deficits beyond frank loss of tissue. J. Orthop. Res. 33, 40–46 (2015). - PubMed
-
- Cross J. D., Ficke J. R., Hsu J. R., Masini B. D. & Wenke J. C. Battlefield orthopaedic injuries cause the majority of long-term disabilities. J. Am. Acad. Orthop. Surg. 19, S1–S7 (2011). - PubMed
-
- Lin C.-H., Lin Y.-T., Yeh J.-T. & Chen C.-T. Free functioning muscle transfer for lower extremity posttraumatic composite structure and functional defect. Plast. Reconstr. Surg. 119, 2118–2126 (2007). - PubMed
-
- Lin S.-H., Chuang D. C.-C., Hattori Y. & Chen H.-C. Traumatic major muscle loss in the upper extremity: reconstruction using functioning free muscle transplantation. J. Reconstr. Microsurg. 20, 227–235 (2004). - PubMed
Publication types
MeSH terms
Grants and funding
LinkOut - more resources
Full Text Sources
Other Literature Sources
Medical